Expert comment – Finding SuperNEMO and solving the mystery of the origin of matter in the universe
Departments 13th December 2017
The Savoy region of France is best known for its fir-lined ski slopes and picturesque Alpine villages. Less known is the fact that, deep beneath some of these slopes, scientists are investigating one of the greatest mysteries in physics: the origin of matter.
The Fréjus road tunnel in the region carries traffic between the French town of Modane and the Italian town of Bardonecchia. Take a drive through the tunnel, and you might just notice – at the mid-point – an unassuming green door in the tunnel wall. This sturdy metal door separates the stifling, diesel-infused air of the road tunnel from the clean, controlled atmosphere of the Laboratoire Souterraine de Modane, Europe’s deepest underground laboratory which is home to a particle physics experiment called SuperNEMO.
The SuperNEMO detector, around six metres long, four metres high and three metres wide, sits in a tightly controlled clean room to protect it from contamination by the minute amounts of natural radioactivity present in dirt and dust. The mountain itself provides protection from the cosmic rays that continuously bombard the surface of our planet. Such protection is needed, since the job of SuperNEMO is to watch over seven kilograms of selenium and search for one of the rarest forms of radioactivity there is: double-beta decay.
All radioactive elements are unstable and decay (split up) to a stable state due to changes in the atomic nucleus (which consists of protons and neutrons). Double-beta decay is a process by which two neutrons in a selenium nucleus simultaneously decay into protons, while emitting two electrons and two particles called antineutrinos.
Antineutrinos are an example of “antimatter”. All matter particles have antiparticle versions of themselves – nearly identical but with opposite charge. When a particle and an antiparticle meet, they annihilate in a flash of energy.
Enigmatic particles
Antineutrinos are puzzling. Take the way they spin, for instance. Many particles spin as they travel, but neutrinos only seem to spin one way. All neutrinos spin anticlockwise as they travel – and all antineutrinos spin clockwise. We have no idea why this is the case.
Then there is their mass: neutrinos are many, many times lighter than any other particle with mass – so much lighter that we have not yet been able to directly measure their tiny mass. The neutrino is an outlier among particles – and when scientists see outliers, we can’t help but suspect there is some deeper meaning behind the inconsistency which could reveal profound truth about the laws of nature. The seeds of a theory to explain the neutrino’s many eccentricities lie in a relatively mundane observation: unlike other particles, the neutrino has no electric charge.
But with no electric charge, how does the antineutrino differ from the neutrino? There definitely is some difference. The kinds of neutrinos and antineutrinos SuperNEMO is looking at are of the so-called electron type. When the neutrinos interact with matter they produce negatively charged electrons, but when the antineutrinos interact with matter they produce positively charged positrons, the electron’s antiparticle. But before the neutrino or antineutrino interacts, how does it know which one it is?
This profound question led the Italian physicist Ettore Majorana to consider whether the neutrino and the antineutrino could in fact be exactly the same particle, just spinning in opposite directions.
If the antineutrinos created in the double-beta decay that SuperNEMO is looking for have the ability to behave like neutrinos, then just occasionally one of them might do that. That would mean you had an antineutrino and a neutrino next to each other – which would mean they could annihilate each other. Should that happen, the two electrons produced in the double-beta decay would get an extra kick of energy from the annihilation – and that is what SuperNEMO is looking for: a tiny kick of energy that would require us to rethink how matter and antimatter are related.
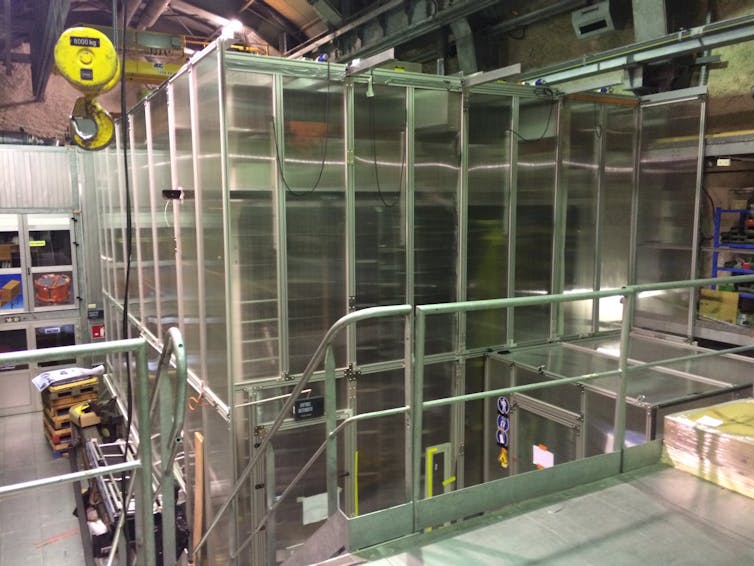
Patience is the key to this search. The half-life of double-beta decay in selenium – that’s the time you’d have to wait before one atom has a 50% chance of having decayed – is 1020 years. That’s a 1 with 20 zeros after it: take the lifetime of the universe and add another ten zeros. And even when a double-beta decay happens, the chance that the two antineutrinos annihilate is tiny – if it even happens at all. We make up for that by having a lot of selenium atoms in our detector, but still we are looking out for only one or two such decays every year.
The origin of matter
If we observe such a radioactive decay we would have to rewrite the successful Standard Model of Particle Physics. This would be a huge discovery in itself. The Standard Model contains strict rules, called conservation laws, about what can and cannot happen in particle decays and interactions. If our two antineutrinos annihilate (because one of them behaved like a neutrino at the time), then the double-beta decay would produce two matter-like electrons and no antimatter to balance them out. That’s not allowed in the Standard Model, which requires that matter and antimatter are always produced in equal amounts.
This brings us to one of the most profound questions of physics: why is there more matter than antimatter in the universe? You might think we already know the answer to that: the Big Bang produced all the matter. Well, yes it did, but it should have also produced an equal amount of antimatter. So why did all the matter and antimatter not annihilate each other to leave nothing but a sea of light?
If the neutrino and antineutrino are indeed the same particle, the resulting revised Standard Model would allow you to add more of these neutrino-like particles into your model. Some of these neutrino-like particles might be heavy rather than light; and I mean very heavy – so heavy that the Large Hadron Collider hasn’t been able to produce them, and so heavy in fact that they were only common in the hot, dense conditions of the very early universe.
Since this revised Standard Model has a mechanism to break the symmetry between matter and antimatter, these super-heavy neutrinos also have the ability to “choose” to decay into matter over antimatter, providing the early universe with the extra matter we now see. If it didn’t, all the matter and antimatter would have annihilated each other and there would be no stars, the planets, and us.
So if you are ever in the Savoy region of France, enjoying some aprés-ski after a day on the slopes, spare a thought for the SuperNEMO detector – and the particle physicists like me, deep below you, waiting patiently for that radioactive decay that just might explain how you got to be there.
Words – Justin Evans, Senior Lecturer in Physics, University of Manchester
This article was originally published on The Conversation. Read the original article.
matterparticle physicsPhysicsPhysics and AstronomySpaceSuperNEMO